What if Aesop’s Tortoise Were Smarter?
Rethinking the chromatography component of routine LC-MS bioanalysis
At a Glance
- Increasingly often, we need to measure small or low-concentration analytes like biomarkers in complex biological samples
- One common way to do so is LC-MS – but it’s not ideal because slow, inefficient liquid chromatography results in poorly utilized mass spectrometry
- Newer techniques like mobile affinity sorbent chromatography can improve the efficiency of the first step
- There are a number of efficient chromatography options; all that remains now is to clear the financial obstacles to improving LC-MS
The Problem
There is a growing need for routine analysis of small numbers of analytes in complex biological samples – and the world is increasingly turning to liquid chromatography-mass spectrometry for answers despite the challenges of low throughput and high cost per sample.
Background
There is great interest today in measuring small sets of biomarkers in biological samples as a means to assess biological function, health, disease, and treatment efficacy (1)(2), which is in turn putting new demands on the separation sciences.How can we separate, identify, and quantify relevant markers in samples that contain 10,000 other components within minutes – millions of times annually? Many believe LC-MS will play a role in
this endeavor.
A major problem in routine analysis via LC-MS is that the LC column captures most of the substances in a sample; all of which elute into the MS over the course of the separation. With samples containing 10,000 or more components (see Figure 1), analytes and non-analytes will co-elute (3), making analyte differentiation difficult. Additionally, co-eluting non-analytes cause ion suppression, add background noise in spectra, and produce fragment ions of mass similar to analytes. Although MS is capable of millisecond analyses, analytes elute from LC columns over long periods of time; throughput is low, and elution times vary with instrument type, temperature, column lot, and column aging. As a consequence, the MS must continuously collect and examine huge amounts of useless spectral data to assure that analyte data is captured. Clearly, the MS is being poorly utilized.
The scenario brings to mind Aesop’s tortoise and the hare; in an LC-MS/MS version of the fable, chromatography would be Mister LC Tortoise and the speedster would be Mister MS Hare. One realizes that, although illogical, this fable is played out millions of times annually in routine analyses. MS is fast and underutilized while LC trudges along. In the real world, LC Tortoise would have to be cleverer to survive – insisting on rules that include i) allowing him to finish in a few steps, ii) greater exploitation of his unique skills, iii) a course that is difficult for MS, and iv) requiring MS to do more work to finish. LC could dupe MS into accepting this rule change by telling him: “These new rules will make the race a little stressful but it will be over quickly, proving how fast you are. It will be more like a sprint than a marathon, so falling asleep and waking up in time to win will no longer be a problem for you!” In actual fact, LC has skewed the rules far in his favor.
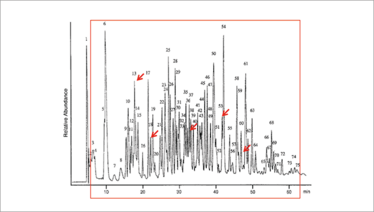
Figure 1. An illustration of problems encountered in LC-MS analysis of small numbers of analytes in complex biological samples. Red arrows show targeted analyte elution positions while the red box contains analytes that enter the mass spectrometer. Adapted from Reference 3 (highlighting added).
The solution
There is clearly a problem with the way LC is being used in routine analytical applications. Although MS can resolve the highlighted analytes (see Figure 1) a thousand times faster than LC, it cannot rid the effluent of non-analytes. The primary function of the LC should be to provide the MS exclusively with analytes of interest (devoid of non-analytes), rather than dividing complex mixtures into hundreds of fractions.
The problem could be circumvented if the small number of recurring analytes in the sample were structure-specifically selected and eluted concurrently in a cluster – unretained and relatively pure in a first chromatographic peak, and ahead of solvents and non-analytes – such that analytes could be rapidly transferred to an MS together (see Figure 2). The LC would be delivering a small group of highly purified, structure-specifically selected analytes to the MS for fractionation, identification, and quantification while discarding non-analytes. Being able to achieve this in two steps within minutes would have relatively large ramifications.
Structure-specific selection of analytes from complex mixtures is something that MS cannot do, whereas the LC could do so in minutes with high reproducibility. Moreover, nothing of analytical value would be eluted into the MS beyond the first chromatographic peak. The major work of the LC would be finished after delivering a single fraction to the MS. Non-analytes could be discarded by valve switching. Moreover, it would enable reduction of ion suppression, suppress background noise, and diminish fragment ion overlap in the MS. Structure-specific selection by the LC would be a critical component of the analysis, but the MS would be doing most of the work. The tortoise would be doing a critical thing MS cannot – quickly separating a small group of targeted analytes from the thousands of non-analytes in samples. Moreover, there would be no reason to fractionate all the non-analytes in a sample as shown in Figure 2, saving a huge amount of time. The tortoise would have crossed the finish line in two steps while the MS must do a large amount of work to finish. I think Aesop might have liked this “clever Tortoise” version of his fable...
We have achieved rapid, group-specific selection of a small number of analytes from complex mixtures as suggested above by developing a new type of chromatography. We call it mobile affinity sorbent chromatography (MASC) and first presented it at HPLC 2016 in San Francisco.
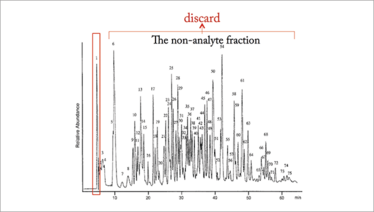
Figure 2. An MASC solution to the problem illustrated in Figure 1. The red box is where affinity selected analytes would coelute. All other substances would be discarded before entering the mass spectrometer. Adapted from Reference 3 (peaks highlighted in Figure 1 have not actually been removed from Figure 2).
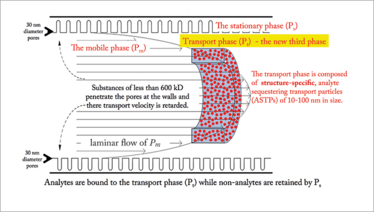
Figure 3. An illustration of the mobile affinity sorbent chromatography (MASC) model, demonstrating the three phases involved in separation processes. The function of the transport phase (Pt) is to sequester and accelerate the elution of analytes while that of the stationary phase (Ps) is to bind and retard elution of non-analytes. The mobile phase regulates partitioning between these two phases. This rapidly separates analytes from non-analytes.
LC separations have long been based on differential partitioning of substances between two immiscible phases; one being an analyte transporting mobile phase (Pm) and the other a stationary phase (Ps) through which Pm is flowing. MASC is different, because a third, structure-specific analyte sequestering transport phase (Pt) is added to the conventional two phase LC system (see Figure 3). The function of this new transport phase is to i) sequester analytes of interest with high selectivity and affinity, based on their structure, ii) preclude their interaction with Ps, and iii) transport them though the column unretained. Pm still plays the role of mediating partitioning and transport.
The Pt used in our early MASC experiments is composed of 20-80 nm hydrocolloids with coupled affinity selectors. These analyte-sequestering transport particles (ASTPs) are larger than most substances in plasma while being sufficiently small to pass readily between the particles in a size exclusion chromatography (SEC) column or restricted access media (RAM) system. Single-structure specific-affinity selectors (Sa) were a component of each ASTP (see Figure 4) and, in our early studies, were antibodies (Ab) with an analyte association constant typically exceeding 106. Subsequent to association with the Ab, analytes are transported through MASC columns without desorption from the ASTP (4).
ASTP particles of ~60 nm eluted in the void volume of a 30 nm pore diameter SEC column designed for the separation of water soluble substances (see Figure 5). Non-analytes of less than ~400 kilodaltons (kDa) enter the SEC pore matrix and elute after ASTPs; the retention time of ASTPs being in the range of a minute based on column dimensions and flow rate. Beyond enabling ASTP:analyte elution in the column void volume, a second advantage of MASC with an SEC column is that non-analytes have a short retention time, in contrast to the reversed phase chromatograms in Figure 1 and 2.
MASC was achieved in two ways; either by continuously adding ASTPs to Pm or by pre-equilibration of ASTPs with samples followed by injection of a small aliquot of the sample-bearing analyte:ASTP complexes. The latter of these two separation modes is referred to as zonal MASC in view of the fact that the small zone of analyte:ASTP complex acts like a short column from which weakly adsorbed substances are being continuously stripped as the particles move through the SEC column. Zonal MASC has multiple advantages, with the most important being that analytes bind to the ASTP before introduction into column, circumventing the need for in-column association of analytes with the Pt, which minimizes band spreading. Zonal MASC also minimizes antibody consumption and results in non-specifically bound substances being actively removed from equilibrations with ASTPs. Finally, fresh sorbent can be used in each analysis; minimizing carryover – equivalent to using a new affinity chromatography column for each analysis.
Analyte detection by MS in MASC is best achieved by dissociation of the analyte:ASTP complex after elution from the LC column. When the affinity selector is an antibody this entails antibody denaturation by heating or addition of an acidified organic solvent. With electrospray ionization-mass spectrometry (ESI-MS) high-temperature gas in the nebulizer spray was used to denature antibodies and desolvate the products before transport into the MS as seen in the detection of carbamazepine (see Figure 5).
Beyond the solution
The intent of the discussion above was to direct attention to three key facts:
- The best solution to a problem may not be the most widely used,
- The LC component of LC-MS for routine analysis is low-throughput and unoptimized,
- Column parameters, such as particle size, theoretical plates, and peak capacity, are not always the dominant issues in an LC separation.
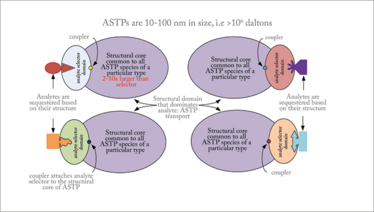
Figure 4. An illustration of the components in an analyte sequestering transport particle (ASTP).
Although enormous gains have been made in structure-specific selection technology coupled to MS, adaptation in routine LC-MS has been disappointing. Structure-specific selection of analytes for MS analysis is actually an old technique – immunoaffinity assays using MS detection were first described in 1991 (6), followed by a host of MS-assisted assay methods ranging from mass spectrometric immunoassays (MSIA, (7), affinity-MS (8), and probe affinity mass spectrometry (PAMS, (9) to immunoMALDI (iMALDI, (10), surface-enhanced laser desorption ionization-TOF (SELDI-TOF, (11), and surface-enhanced affinity capture (SEAC, (12). These strategies all exploited either affinity chromatography with ESI-MS or affinity selection on MALDI plates as a means to simplify the purification of specific analytes – and they worked beautifully.
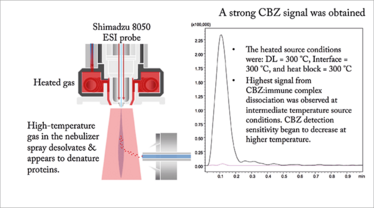
Figure 5. The inlet system used in ESI-MS analysis of carbamazepine.
And yet, even with all of these powerful hyphenated-tools, reverse phase chromatography (RPC) still dominates sample preparation in routine LC-MS analysis of complex biological samples. As the LC-MS version of Aesop’s fable suggests, the dominance of this old method is illogical. MASC with analyte-sequestering transport particles is simply another in a long list of structure-specific selection epiphanies (albeit one of the more powerful).
The future?
Scientists often seek – or expect scientific explanations for – puzzling phenomena such as the LC-MS conundrum noted above in routine analysis. But perhaps the dilemma is not of scientific origin. Clearly, the issues noted above obstruct the delivery of high-throughput, inexpensive diagnostics to millions of human subjects; removing these obstacles would be of massive value. So why hasn’t the LC-MS/MS enigma in routine analysis been addressed?
It is often overlooked by the scientific community that economics towers above the other “omics.” Finding the “right time” and mode of delivering a new technology often requires large investments. One skill of the investment community lies in guessing (or betting on) those elements and solutions that would provide the greatest return on investments, along with providing the requisite capital to back their bet. The manner and timeline in which a routine analysis revolution is triggered will more likely be a function of economic drivers than scientific issues.
As an afterthought, the “clever tortoise” version of Aesop’s fable should probably have included a venture capital investor who would finance the contest and declare a winner based on investment returns. Looking at this as a sporting event with betting and a P&L bottom line is perhaps much more exciting than increasing LC-MS throughput, decreasing ion suppression, and eliminating background noise in spectra.
- SKG Grebe and RJ Singh, “Clinical peptide and protein quantification by mass spectrometry (MS)”, TrAC, ahead of print (2016).
- A Zhang et al, “Serum proteomics in biomedical research: a systematic review”, Appl Biochem Biotechnol, 170(4), 774-786 (2013).
- Q Luo et al, “ MicroSPE-nanoLC-ESI-
- MS/MS using 10-μm-i.d. silica-based monolithic columns for proteomics”, Anal Chem, 79 (2), 540-343 (2007).
- F Regnier and Jinhee Kim, “Near-continuous separation, purification and analysis of body fluid samples utilizing affinity transport particles in conjunction with size-exclusion chromatography and mass spectrometry”,
- PCT Int Appl, WO 2016019166 A1 (2016).
- X Feng et al, “Research progress of matrix effect in determining blood concentration by LC-
- MS/MS”, Chin J New Drugs [ZDB], 24 (13), 1488-1492 (2015).
- JE Alexander et al, “Characterization of posttranslational modifications in neuron-specific class III β-tubulin by mass spectrometry”, Proc Natl Acad Sci USA,
- 88(11), 4685-4 (1991).
- D Nedelkov, “Mass spectrometry-based immunoassays for the next phase of clinical applications”, Expert Rev Proteomics, 3(6), 631-640 (2006).
- DI Hayes and KB Tomer, “Direct analysis of affinity-bound analytes by MALDI/TOF MS”, Anal Chem, 66, 2609-2613 (1994).
- AH Brockman and R Orlando, “Probe-immobilized affinity chromatography/mass spectrometry”, Anal Chem, 67(24),
- 581-5 (1995).
- H Yan et al, “Noncovalent antibody immobilization on porous silicon combined with miniature solid-phase extraction (SPE) for array based ImmunoMALDI assays”, Anal Chem, 83(12), 4942-4948 (2011).
- M Merchant and SR Weinberger, “Recent advancements in surface-enhanced laser desorption/ionization-time of flight mass spectrometry”, Electrophoresis, 21(6), 1164-1177 (2000).
- H Kuwata et al, “Direct detection and quantification of bovine lactoferrin and lactoferrin fragments in human gastric contents by affinity mass spectrometry”, Adv Exp Med Biol, 443, 23-32 (1998).
Fred Regnier is J.H. Law Professor of Chemistry Emeritus at the Department of Chemistry, Purdue University, Lafayette, USA.